Science Highlights, August 5, 2015
Awards and Recognition
Accelerator Operations and Technology
Ultrafast laser driven shocks allow observation of initial chemistry behind a shock front
Earth and Environmental Sciences
Model evaluates heat-generating nuclear waste disposal in bedded salt formations
Materials Physics and Applications
Neutron scattering showcased as Editor’s Pick in special Biointerphases issue
Awards and Recognition
Darleane Hoffman and Wojciech Zurek receive Los Alamos Medals
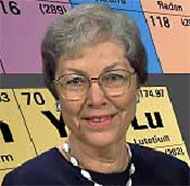
Darleane Hoffman
Darleane Hoffman and Wojciech Zurek are 2014 Los Alamos Medal recipients, the highest honor that the Laboratory bestows. Their achievements are described in the following paragraphs.
Hoffman is an expert in the science of actinide and transactinide elements, separation science, radiochemistry, and nuclear chemistry. Her contributions to actinide science span more than 60 years of investigations into the chemistry and nuclear physics of actinide elements. She has also supported science related to DOE missions through leadership in science and programs, mentoring, and service to the nation.
Hoffman began her prestigious career at Los Alamos Scientific Laboratory in 1953 as a staff member and project leader in the former Field Testing Division. Originally hired to develop rapid, efficient plutonium analyses for nuclear test debris, she served as a radiochemist at the Laboratory for over 25 years. She was personally instrumental in the development of procedures for separating f-elements from complex mixtures and matrices. Methods she created for separating plutonium and the actinides became the basis for analyzing nuclear test debris and are currently used in the national security community (including at LANL) for programs in nonproliferation and treaty verification. Her work in radiochemistry in the test program contributed significantly to the understanding of nuclear weapons performance and generated experimental data that are still used to validate and improve weapons codes.
Hoffman’s separation and actinide science expertise led to fundamental and applied research accomplishments. She and co-workers isolated and purified approximately 8 x 10-15 g of naturally occurring plutonium-244 from about 85 kg of Precambrian bastnasite ore, a feat characterized by the late Glenn Seaborg as “an experimental tour de force”. Until this seminal work, scientists believed that transuranium elements did not exist in nature. The research required exquisitely detailed and careful separations to extract the plutonium from thorium and the abundance of rare earth elements for mass spectrometric analyses on the resulting plutonium fraction. Nature published the research. Intrigued by the nuclear properties of elements, Hoffman sought new heavy elements in nuclear test debris. Before these isotopes could be prepared for further study, they had to be carefully isolated and purified. She and co-workers conducted groundbreaking studies of the fission properties of fermium-257 isolated from nuclear tests and from targets irradiated at Oak Ridge National Laboratory. Her observation in fermium isotopes of enhanced symmetric mass division, a form of spontaneous fission that results in two daughter nuclei nearly equal in mass, led to the revision of fission theories. In applied research, her team conducted a multi-year project to examine the migration of fission products and actinides from the Cambric nuclear explosion at the Nevada Test Site. These early studies of radionuclide migration in underground environments have helped scientists formulate methods for safe storage of nuclear waste and its isolation from the environment.
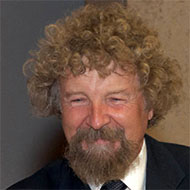
Wojciech Zurek
In 1979, the Lab appointed Hoffman to lead the Chemistry and Nuclear Chemistry (later called Isotope and Nuclear Chemistry) Division. She charted the course of new programmatic directions in heavy elements and radionuclides related to fundamental research, predictive understanding of radionuclide behavior in proposed nuclear waste repositories, environmental behavior of radionuclides, and medical radioisotopes for imaging and therapy. Hoffman was named a Laboratory Fellow and continues to provide service to the Laboratory as a consultant.
Hoffman spent sabbaticals at the Institute for Atomic Research in Norway and as a Guggenheim Fellow at Glenn Seaborg’s lab at Lawrence Berkeley National Laboratory studying the heaviest elements. In 1984, she became a professor in the Department of Chemistry at the University of California, Berkeley, where she remains. Her “atom-at-a-time” chemistry (she coined the term in 1993) made possible the study of heavy elements with half-lives of less than one minute. Her group investigated the chemistry of element 104 (rutherfordium) and was the first to investigate the aqueous chemistry of 105 (dubnium), 106 (seaborgium), 107 (bohrium), and 108 (hassium). She and her colleagues confirmed the discovery of element 106, which was named seaborgium for Nobel laureate Glenn Seaborg. Hoffman also served as the charter director and now a senior research adviser at Lawrence Livermore National Laboratory’s Seaborg Institute for Transactinium Science.
Hoffman’s awards include: American Physical Society Fellow, American Association for the Advancement of Science Fellow, American Chemical Society Fellow, U.S. National Medal of Science recipient, American Chemical Society’s Priestley Medal, Women in Technology International Hall of Fame, Francis P. Garvan-John M. Olin Medal, Sigma Xi Proctor Prize for Scientific Achievement, and numerous recognitions from foreign countries and scientific associations. She has published some 280 papers in refereed journals with over 4000 citations.
Zurek has made pioneering and seminal contributions to the foundations of quantum mechanics and to quantum information science that have changed the course of this field internationally. The Laboratory honored him for his decoherence research that has established the bridge between the quantum and classical realms, and his work in quantum cryptography relevant to national security.
Zurek joined the Laboratory as an Oppenheimer Fellow in 1984.
He is a leading world authority on quantum theory, especially decoherence and the physics of quantum and classical information. The relation between classical and quantum physics had been a puzzle ever since the introduction of quantum wavefunctions. For over six decades “wavefunction collapse” during measurements was the practical description. It was unsatisfactory because it presumes pre-existing classical domain and leaves unclear what is a measurement and what is not. Zurek’s analysis of the consequences of entanglement of quantum states with their environments affords a smooth transition from the quantum to classical realm. As a system entangles with more and more of the environment, more and more quantities would have to be simultaneously followed to observe a fully quantum behavior. States that are stable in spite of the environment define the classical domain, a quasiclassical subset of all possible quantum superpositions – quantum states. The loss of phase coherence causes this environment-induced superselection, leading to effectively classical behavior. As a result of his research, one can now derive classical physics from quantum theory, much as one takes non-relativistic limit in relativity. The smooth quantum-to-classical transition provides sophisticated and satisfactory answers to many fundamental questions and explains why macroscopic objects (that are hard to isolate) are classical.
Zurek also demonstrated (with William Wootters and published in Nature) that an unknown quantum cannot be cloned, which is now widely known as the No-Cloning Theorem. The theorem is key to quantum cryptography and to quantum information processing, including quantum error correction, a subject to which Zurek has also contributed significantly.
Zurek’s awards include: Alexander von Humboldt Prize, selection as a Phi Beta Kappa Visiting Lecturer, Marian Smoluchowski Medal (highest prize of the Polish Physical Society), Albert Einstein Professorship Prize of Ulm University, and the Order of Polonia Restituta (similar to the Knighthood in the United Kingdom). He is a Fellow of the American Physical Society and Los Alamos National Laboratory. Zurek has authored about 250 papers (approaching 18,000 citations).
The Laboratory established the Los Alamos Medal in 2001 to honor those who have contributed to the Laboratory at the highest level. The award recognizes individuals who have made
- a contribution that changed the course of science
- a major enhancement of the Laboratory’s ability to accomplish its mission
- a significant impact on Laboratory sustainability and/or established a major direction for the Laboratory and/or the nation
Hoffman and Zurek join past Los Alamos Medal recipients Hans Bethe and Harold Agnew (2001), George Cowan and Louis Rosen (2002), Nerses “Krik” Krikorian (2003), Francis “Frank” Harlow and Conrad Longmire (2004), Keith Boyer and Stirling Colgate (2006), and Robert Cowan and Sig Hecker (2008) in receiving the prestigious recognition. Technical contact: Melissa Robinson
Accelerator Operations and Technology
Ultrafast laser driven shocks allow observation of initial chemistry behind a shock front
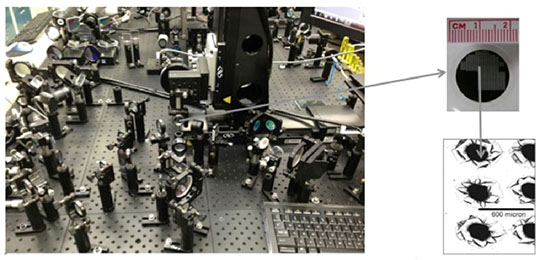
Photo. A tabletop laser system drives hundreds of microscale shock experiments a day with picosecond (ps) synchronization to numerous optical diagnostics.
Nitromethane is one of the simplest and most thoroughly studied explosives, yet very little is known about the shock-induced chemistry that drives detonation. Fast shock-induced chemistry is an essential characteristic of detonation, but this chemistry remains a mystery for explosives in use today. Predictions for the timescale of shock induced chemistry in detonating nitromethane range from ten nanoseconds for empirical reactive burn models to tens of picoseconds for quantum molecular dynamics models. Until recently, there were no experimental means to directly probe this discrepancy of three orders of magnitude variation in kinetic predictions. Now, a Los Alamos team has performed ultrafast shock chemistry experiments demonstrating that substantial chemical reactions occur in tens of picoseconds near detonation conditions in nitromethane. The Journal of Physical Chemistry A reported the research.
Ultrafast experiments can access chemical initiation information of shocked materials that is unattainable in traditional plate impact experiments. Lab researchers have been developing tabletop ultrafast laser driven shock capabilities for over 15 years to observe the chemical events that support detonation (Photo).
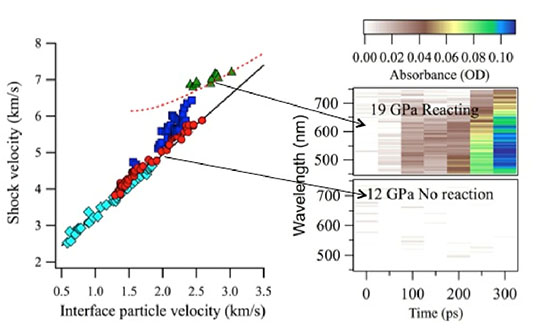
Figure 10. Shocked nitromethane exhibits chemical reactions as seen through deviations from the black solid line on the Hugoniot plot (left) and through the transient absorption of the products (right). On the left plot, diamonds are plate impact data previously recorded on nanosecond to microsecond time scales. The red circles, blue squares, and green triangles are ultrafast laser shock driven data measured in less than 300 ps. The red circles are not reacting in the first 300 ps, the blue are beginning to react over 300 ps, and the green are reacted within tens of picoseconds. The black curve is an unreactive reference Hugoniot, and the red dashed line is the predicted product curve.
A LANL team has used these capabilities to measure the properties within the first 300 ps behind the shock front in nitromethane. Figure 10 summarizes the interferometric and transient absorption results. The observed reactions at 19 GPa are significantly faster than predicted by reactive burn models of nitromethane that have been parameterized to fit a broad range of data on detonation wave profiles and shock to detonation transitions. However, the measured interferometry data agree with the properties of the predicted products, the red dashed curve. In contrast, the observed reaction rates are similar to those predicted by quantum molecular dynamics. The chemical species appear to be different because the optical properties observed disagree with predictions. As the experimental measurement specificity improves, researchers could test the details of the theory and simulations even more stringently.
The optical measurements of shocked chemistry at picosecond timescales set the stage for time- resolved x-ray chemical measurements that could be performed at a facility such as MaRIE (Matter-Radiation Interactions in Extremes), the Laboratory’s proposed facility for materials studies at the mesoscale. Eventually, iteration between improvements in theory and experiment will converge upon a complete picture of the essential chemistry of detonating nitromethane and other explosive materials.
Reference: “Ultrafast Chemical Reactions in Shocked Nitromethane Probed with Dynamic Ellipsometry and Transient Absorption Spectroscopy,” Journal of Physical Chemistry A 118, 2559 (2014); doi: 10.1021/jp4125793. The team includes: Kathryn Brown, Cynthia Bolme, and Shawn McGrane (Shock and Detonation Physics, M-9); and David Moore (Explosives Science and Shock Physics, M-DO).
NNSA Campaign 2 (LANL Project Leads Margo Greenfield and Dan Hooks) currently funds the research; the Laboratory Directed Research and Development (LDRD) program initially sponsored the work. The research supports the Laboratory’s Nuclear Deterrence mission area and the Materials for the Future science pillar by furthering understanding of shock-induced chemistry toward the long-term goal of achieving predictive reactive burn modeling of high explosives. Technical contact: Shawn McGrane
Bioscience
Combined crystallographic and NMR studies of carbonic anhydrase
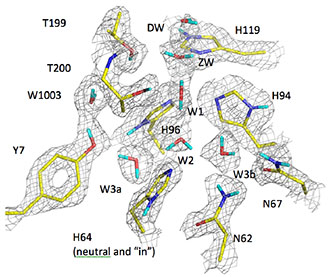
Figure 1. Active site of HCA II enzyme shows crucial Tyrosine 7 (Y7) and Histidine 64 (H64) residues and a well ordered water network that includes molecules labeled DW, ZW, W1, W2, W3a, W3b and W1003.
Proton transfer is a fundamental mechanism at the core of many enzyme-catalyzed reactions. It is exquisitely sensitive to pH, electrostatics, active-site geometry, and chemistry. Carbonic anhydrases (CAs) are ubiquitous enzymes that catalyze the interconversion of carbon dioxide (CO2) and hydrogen carbonate (HCO3). Human CAs are involved in a great variety of physiological processes: respiration, ureagenesis, gluconeogenesis, cerebrospinal fluid production, and general acid/base homeostasis. The CA enzyme has evolved a fast and efficient way to conduct protons through a combination of hydrophilic amino acid side chains that coordinate a highly ordered hydrogen-bonded water network. LANL scientists and collaborators have studied the structure of CA using a combination of neutron crystallography and nuclear magnetic resonance (NMR) to gain a better understanding of proton transfer and how subtle changes in ionization and hydrogen-bonding interactions can modulate enzyme catalysis. Proceedings of the National Academy of Sciences published the research.
In the Human Carbonic Anhydrase II (HCA II) enzyme, a proton derived from water bound to the zinc atom in the active site is transferred to the bulk water surrounding the enzyme. The proton transfer network involves several highly ordered water residues, and amino acid residues tyrosine 7 (the 7th amino acid in the molecule) and histidine 64 (the 64th amino acid) from the enzyme. Histidine 64 becomes protonated, swings out into the bulk water, and releases the proton.
To understand the process, the authors probed the protonation state of all tyrosine amino acids in HCA II and measured their pKa (acidity constant) in solution, with particular focus on tyrosine 7. They combined these studies with analysis of new neutron crystallographic structures, which enable direct visualization of hydrogen atoms in proteins. The information provides insight into the effect of pH on important active-site residues. The team measured NMR spectra from pH 5.4 - 11.0. Identification of all eight tyrosines in HCA II allowed interrogation of their individual behaviors in solution and a comparison of the results with the crystal structures. The data revealed that tyrosine 7 has a pKa three units lower than the other tyrosines and matches that of histidine 64 in order to participate in the proton transfer.
This is the first example demonstrating the powerful combination of NMR spectroscopy and neutron crystallography to gain insight into the electrostatics of a protein active site and how such electrostatics affect the charged states and hydrogen-bonding of amino acid residues. The results show that the protonation states of histidine and tyrosine residues are sensitive to both pH and the presence active site zinc atom. These factors change how histidine and tyrosine residues hydrogen-bond to water and other amino acids. The research shows that it is possible to study these changes directly and at high resolution.
Reference: “Joint Neutron Crystallographic and NMR Solution Studies of Tyr Residue Ionization and Hydrogen Bonding: Implications for Enzyme-mediated Proton Transfer,” Proceedings of the National Academy of Sciences 112, 5673 (2015); doi: 10.1073/pnas.1502255112. Authors: Ryszard Michalczyk, Cliff Unkefer, and John-Paul Bacik (Bioenergy and Biome Sciences, B-11); Andrey Kovalevsky and Zoe Fisher (formerly B-11); collaborators from Forschungszentrum Jülich GmbH, Technische Universität München, Oak Ridge National Laboratory, and University of Florida.
Researchers prepared amino acids for NMR studies at the LANL Stable Isotope Resource. Pete Silks (B-11) provided stable isotope labeled tyrosine used in some labeling experiments. The research used two instruments – X-ray Diffractometer and 700 MHz NMR – that the Principal Associate Directorate for Science, Technology and Engineering (PADSTE) purchased as an institutional investment. The DOE Office of Science sponsored the Protein Crystallography Station at the Los Alamos Neutron Science Center, and a LANL Laboratory Directed Research and Development (LDRD) Early Career grant funded research. The work supports the Lab’s Global Security mission area and the Science of Signatures science pillar via the elucidation of the structure and mechanisms of important human enzymes. Technical contact: Ryszard Michalczyk
Earth and Environmental Sciences
Model evaluates heat-generating nuclear waste disposal in bedded salt formations
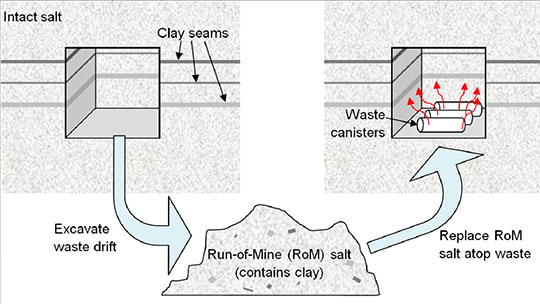
Figure 2. Schematic of the in-drift waste disposal concept.
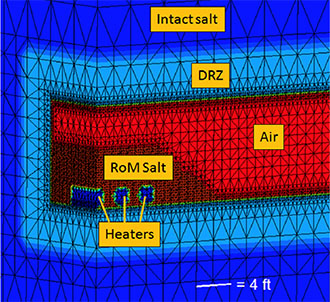
Figure 3. Numerical mesh showing the zones of the model: intact salt, damaged rock zone (DRZ), air, run-of-mine (RoM) backfill, and the heated waste canisters.
Because bedded salt formations contain clay and hydrous minerals that release water when heated, Los Alamos researchers developed a model to include this source term for fluid around high-temperature waste. The scientists produced an experimentally-based model for hydrous mineral dehydration and implemented it with the Finite Element Heat and Mass transfer (FEHM) numerical simulator. FEHM is a LANL-developed controlled volume finite element code for simulating subsurface multi-phase multi-fluid heat and mass transfer to model non-isothermal fluid flow.
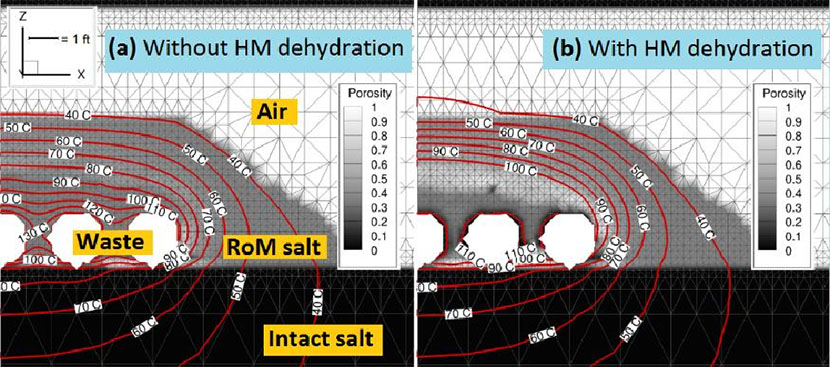
Figure 4. Porosity and temperature after 460 days of heated waste emplacement in crushed run-of-mine (RoM) salt backfill in a generic bedded salt repository, without (a) and with (b) the new hydrous mineral (HM) dehydration model included and an assumed impurity fraction of 10%. With HM dehydration, the simulation shows evidence of heat pipe development, with a flatter temperature gradient, substantial porosity reduction around the waste canisters from evaporating brine, and a high-porosity ring near 100oC where freshly condensed water dissolves solid salt.
The team heated samples from the Waste Isolation Pilot Plant (WIPP) near Carlsbad, NM, to specific temperatures designed to capture a series of dehydration reactions. Water loss of over 20% by weight of hydrous mineral fraction occurred. The additional water in the system could have a significant effect on final temperature, porosity, and saturation. The scientists averaged the data into a stepwise water source term model and implemented it in drift-scale FEHM simulations of heated waste canisters to determine the impact on predicted properties. For some cases, the additional source term of fluid did not significantly affect final results. However, in some combinations of uncertain parameters (initial saturation, maximum capillary suction), the extra water provided by hydrous mineral dehydration affected the onset of a three-phase heat pipe. In a heat pipe, liquid water is vaporized in a boiling region, convects, and diffuses along vapor concentration gradients to cooler regions where it recondenses and flows back towards the heat source by a combination of gravity flow and capillary pressure gradients. If brine re-enters the boiling region, it could vaporize and deposit dissolved salt as a precipitate. This process would contribute to the accumulation of a low-porosity rind around the waste. In these simulations, evidence for heat pipe activity was indicated by reduced porosity around the heaters, a high-porosity region where water vapor condenses and dissolves the run-of-mine (RoM) solids, and a flatter temperature gradient around the canisters. Figure 4 shows simulation results at 460 days after heating by 750 W waste canisters. Including the new hydrous mineral dehydration model produced significantly different temperatures (up to 25oC cooler around the heated canisters) and solid salt redistribution. The authors conclude that the addition of water from hydrous mineral dehydration could make a significant difference in final temperature, moisture, and porosity redistribution. The redistribution of mass, moisture, and temperature at early times may impact the time evolution of salt plasticity and mechanical deformation at longer time scales. Other strongly coupled thermal, hydrological, and chemical processes around heat-generating nuclear waste in bedded salt formations could also be modified by water released from minerals
Reference: “Hydrous Mineral Dehydration around Heat-Generating Nuclear Waste in Bedded Salt Formations,” Environmental Science & Technology 49 (11), 6783 (2015); doi: 10.1021/acs.est.5b01002. Authors: Amy Jordan and Philip Stauffer (Computational Earth Sciences, EES-16), Hakim Boukhalfa and Florie Caporuscio (Earth Systems Observations, EES-14), and Bruce Robinson (formerly in Science Program Office, PADSTE-SPO and currently in ADEP-Program Directors Office, ADEP-PDO).
The DOE Office of Nuclear Energy and Office of Environmental Management funded the work. The research supports the Lab’s Energy Security mission area and the Information, Science, and Technology science pillar through the development of models to examine nuclear waste repository performance scenarios. Technical contact: Amy Jordan
Intelligence and Space Research
Predicting space weather and protecting satellite hardware
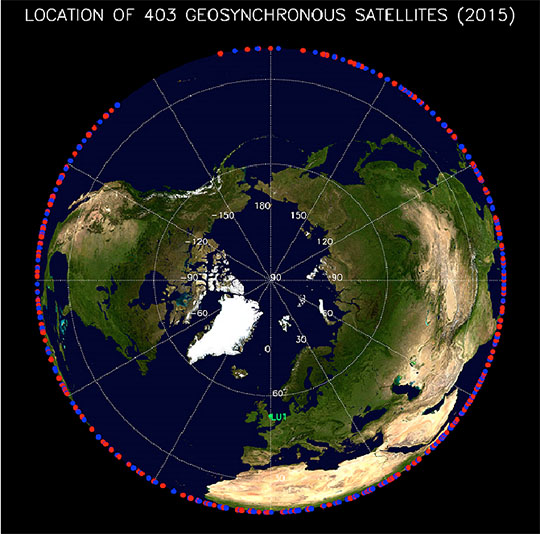
Figure 5. Over 400 military, scientific, and communications spacecraft orbit at GEO. The figure shows the approximate location of these spacecraft - projected to the Earth’s equator.
Geosynchronous orbit (GEO) – roughly 36,000 kilometers above Earth’s surface – is one of the most popular locations for military, scientific, and communications satellites. The 24-hour orbital period at GEO ensures that satellites maintain a fixed location in Earth’s sky. GEO is also the approximate boundary between Earth’s inner and outer magnetosphere, where electromagnetic forces from the two regions control electrically charged particles (electrons and ions) known as plasma. Knowledge and prediction of the environment at GEO is crucial for spacecraft designers and operators because changes in the plasma environment, ultimately caused by the Sun and its solar wind, can interfere with satellite functioning and even lead to satellite failure.
A team of scientists including LANL researchers used 82-satellite years of observations from the Magnetospheric Plasma Analyzer (MPA) instruments on Los Alamos National Laboratory satellites at GEO to create a comprehensive model of how plasma behaves in this region of Earth’s magnetosphere - the most heavily populated orbit for spacecraft traffic. The journal Space Weather published the work. Eos, the leading source for trustworthy news and perspectives about the Earth and space sciences and their impact, highlighted it as a Research Spotlight.
The authors aimed to create a model that could predict the plasma flux environment at GEO in response to rapid changes in geomagnetic and solar activity. The researchers analyzed the vast dataset of electron and ion fluxes from the Magnetospheric Plasma Analyzer. They combined data from seven satellites with observations on solar and geomagnetic activity. The team developed a comprehensive model of the flux of electrons and ions at GEO, for energies between approximately 1 eV and approximately 40 keV, as a function of local time, energy, geomagnetic activity, and solar activity. This energy range encompasses the plasmasphere, the electron plasma sheet, the ion plasma sheet, and the substorm-injected suprathermal tails of both the electron and ion plasma sheets. Satellites on station at GEO encounter each of these populations regularly. The model predicts the fluxes that can cause a buildup of charge on spacecraft materials over a range of energies and time. The new model provides scientific and operational users with prediction of fluxes over a wider range of input conditions than is generally the case with current models. As the model matures, the researchers plan to extend the analysis to predict hazardous fluxes as a function of solar wind speed and magnetic field orientation. These are critical factors that control plasma fluxes at GEO. Such a model would be useful for satellite operators because more than 400 satellites currently reside in geosynchronous orbit (Figure 5). The team has made a beta version of the model freely available at: http://gemelli.spacescience.org/mdenton/
Reference: “An Empirical Model of Electron and Ion Fluxes Derived from Observations at Geosynchronous Orbit,” Space Weather 13, 233 (2015); doi: 10.1002/2015SW001168.
The Los Alamos Laboratory Directed Research and Development (LDRD) program funded the research through the SHIELDS project, which aims to understand, model, and predict Space Hazards Induced near Earth by Large, Dynamic Storms (SHIELDS). This work supports the Lab’s Global Security mission area for space situational awareness and the Science of Signatures science pillar. Technical contact: Vania Jordanova.
Materials Physics and Applications
Neutron scattering showcased as Editor’s Pick in special Biointerphases issue
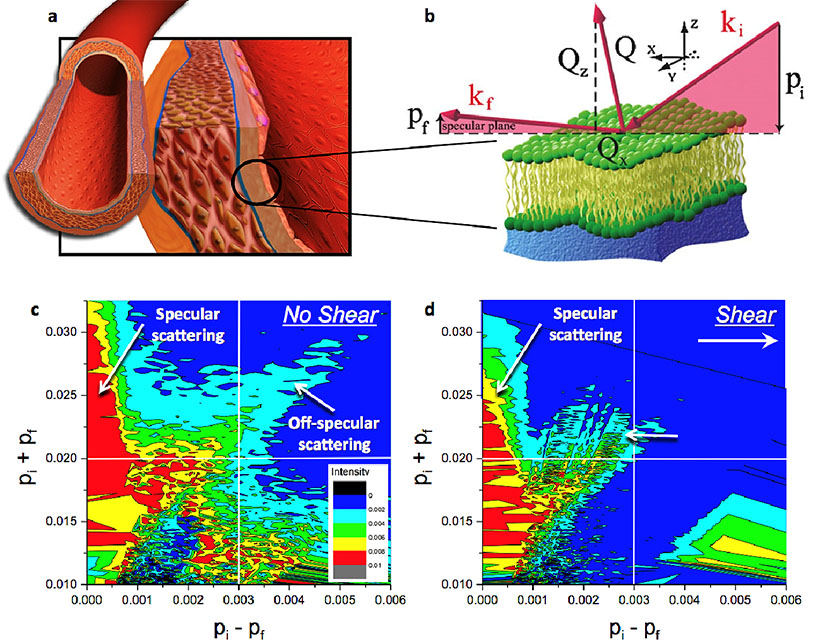
Figure 6. Scientists used neutron scattering at the Lujan Center to study complex bio-interfaces, including the behavior of monolayers of living cells under the stress induced by the flow of liquid mimicking blood. The human vascular system is constantly exposed to blood flow and is inherently reliant on the solid mechanical and fluid mechanical stresses for normal healthy function. (a) The innermost layer of blood vessels is composed of a single monolayer of endothelial cells, the most susceptible to mechanical stress due to the flow of blood. Neutron reflectivity enables researchers to visualize the part of the live cells that is attached to a solid support and to mimic the conditions of the vascular flow during scattering measurements. (b) Specular scattering reveals the mechanism of how the extracellular matrix changes in thickness and density at different temperatures and shear rates. This provides crucial information to understand how endothelial adhesion works and how it might be influenced for biomedical applications. The off-specular data depict changes in the in-plane arrangement of the cells. (c) and (d) show experimental two-dimensional intensity maps of neutrons scattered from endothelial cells at static and shear conditions, respectively. The significant extension of the off-specular signal (right top corner) as (c) compared to (d) indicates more in-plane fluctuations (or disorder) at static conditions. When the cells are under stress due to the flow of liquid, they become more uniformly ordered and aligned.
In research recognized as an Editor’s Pick in a special “in focus” issue of Biointerphases on bio surface analysis, Lab researchers and their colleagues describe recent advances using neutron scattering to study the structure of soft condensed materials. The research included model bio-related systems and in situ investigations of biological interfaces and living cells under mechanical stress.
Understanding these systems at nanometer scales is important for solving many complex biological problems, developing innovative medical treatments, and advancing the development of highly functionalized biomimetic technologies. Examples of the articles include: 1) neutron scattering to study simple systems such as the shear stability of lipid membranes, which has implications in the
design of biocompatible functional coatings; and 2) studies of cancer cell adhesion and response to fluid shear stress, which has implications for understanding the behavior of aggressive brain tumors.
Neutron surface scattering measurements typically examine simple, homogeneous model systems. Studying complex living objects – for example, endothelial or cancer cells in dynamic shear conditions mimicking the flow of blood – presents an extraordinary challenge. The strength of neutron scattering is its non-perturbative nature, the ability to probe large surface areas of buried interfaces with nanometer resolution, and the possibility of manipulating the scattering contrast via isotopic substitutions. These properties enable the examination of structural details not accessible with most other techniques. The information may provide a new way to investigate and understand living tissue in situ. Understanding these complex systems could lead to advances in the treatment of cancer, atherosclerosis, and other disorders associated with the cardiovascular system.
Neutron surface scattering can examine structural changes in soft materials (including those of biological importance) and provide essential structural information to complement the capabilities of MaRIE (Matter-Radiation Interactions in Extremes), the Lab’s proposed facility for materials studies at the mesoscale. Researchers could use these concepts and experimental approaches to conduct new studies that would provide better understanding of biomedical questions, suggest innovative treatment, or advance the development of novel highly functionalized technologies inspired by nature.
Reference: “Analysis of Biosurfaces by Neutron Reflectometry: From Simple to Complex Interfaces,” Biointerphases 10, 019014 (2015); doi: 10.1116/1.4914948. Authors are: Ann Junghans, Saurabh Singh, and Jaroslaw Majewski (Center for Integrated Nanotechnologies, MPA-CINT); Erik B. Watkins (Materials Synthesis and Integrated Devices, MPA-11); Mary Jo Waltman (Bioenergy and Biome Sciences, B-11); Hillary L. Smith (California Institute of Technology); Luka Pocivavsek (University of Pittsburg Medical Center); and Robert D. Barker (Institute Laue-Langevin, France).
This research benefited from the use of the Surface Profile Analysis Reflectometer in the Lujan Neutron Scattering Center at the Los Alamos Neutron Science Center (LANSCE). The DOE Office of Basic Energy Sciences funded the Lujan Center. The work supports the Laboratory’s Science of Signatures and Materials for the Future science pillars through the study of complex and living systems. Technical contact: Jaroslaw (Jarek) Majewski
Materials Science and Technology
Ion implantation enables synthesis of layer-tunable graphene
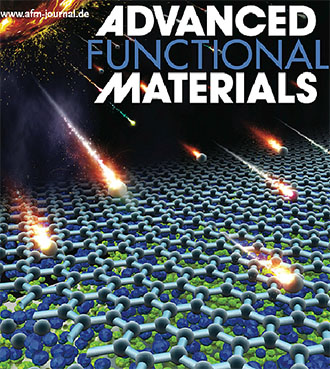
Figure 7. The journal’s back cover features a schematic of the ion implantation process. The method uses ion implantation to introduce a precise dose of carbon atoms (the implanted carbon atoms are depicted as meteors) into the top nickel layer of the nickel/copper bilayer substrate at room temperature (blue depicts nickel atoms and green depicts copper atoms). The implanted carbon ion acts as a carbon reservoir for subsequent synthesis of graphene. Implanted carbon atoms are gradually expelled to the surface and transformed into graphene due to the extremely low solubility of carbon in copper.
LANL’s Ion Beam Materials Laboratory, has the potential to be used in large-scale production lines and speed the application of graphene in nanoelectronics. Advanced Functional Materials published the research and featured a figure from the work on the back cover.
Graphene, a strip of pure carbon one atom thick, is stronger than steel and is an outstanding conductor of electricity and heat. Incorporating this material into real-world applications has proved challenging because the common production method, chemical vapor disposition, is cumbersome, expensive, and toxic. The authors report an ion implantation method that is simpler and offers precise control of graphene thickness, a crucial experimental parameter related to the physicochemical properties of graphene.
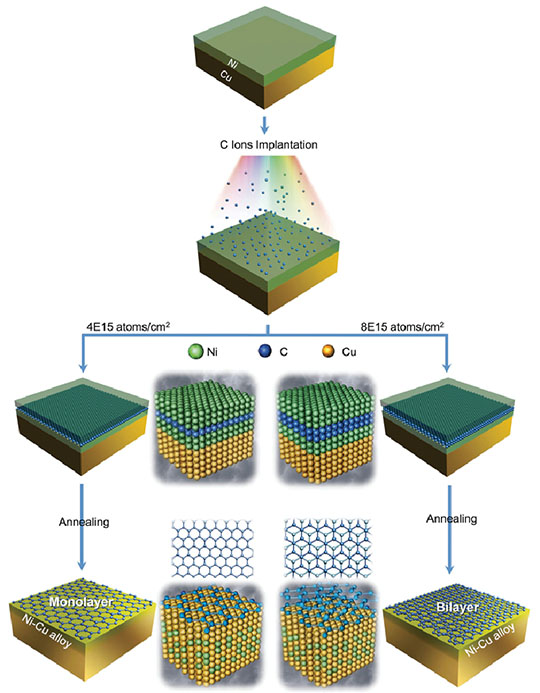
Figure 8. Schematic of the synthesis process to produce single-layer and double-layer graphene by ion implantation. Nickel (Ni) is depicted as green spheres, carbon (C) as blue, and copper (Cu) as yellow. Carbon ions with the predesigned fluence were implanted into the top nickel layer of the Ni/Cu bilayer substrate followed by annealing. The thermal process initiates interdiffusion of Cu atoms and Ni atoms to form the Cu-like alloy. Carbon atoms are expelled from the Cu-like alloy toward the surface, and graphene with the expected layer number is formed on the surface.
Yongqiang Wang (Materials Science in Radiation and Dynamics Extremes, MST-8) used an ion implanter at the Lab’s Ion Beam Materials Laboratory to insert the required number of carbon atoms to form single-layer and double-layer graphene on a copper-nickel substrate. Selection of specific ion energy and fluence enables ion implantation to deliver the exact number of foreign atoms at a precise location within the host material matrix (Figure 8). Implanted carbon atoms expelled during the growth process produce high-quality graphene with the desired number of layers, typically single or double layers. Theoretical calculations confirmed the growth mechanism. This is the first investigation of the use of a dual metal substrate combined with ion implantation for the synthesis of layer-tunable graphene. Because ion implantation is a core technology in microelectronics processing, this method could be implemented into production lines and to expedite the application of graphene to nanoelectronics.
Reference: “Synthesis of Layer-Tunable Graphene: A Combined Kinetic Implantation and Thermal Ejection Approach,” Advanced Functional Materials 25, 3666 (2015), doi: 10.1002/adfm.201500981. Authors: Yongqiang Wang (MST-8) and researchers from the Chinese Academy of Sciences, Lanzhou University, City University of Hong Kong, Shanghai University, East China Normal University, and University at Buffalo - State University of New York.
The Center of Integrated Nanotechnologies (CINT), a DOE nanoscience user program jointly operated by Los Alamos and Sandia national laboratories, provided partial support. The Ion Beam Materials Laboratory is a multi-user research facility with multiple sponsors [DOE Basic Energy Sciences, DOE Nuclear Energy, Laboratory Directed Research and Development (LDRD) program, and the University of California Lab fee research]. The work supports the Laboratory’s Energy Security mission area and Materials for the Future science pillar via advancement of the science to develop materials with properties optimized for specific functions and applications, such as nanoelectronics. Technical contact: Yongqiang Wang
Physics
Measuring the anisotropy in uranium-235 fission
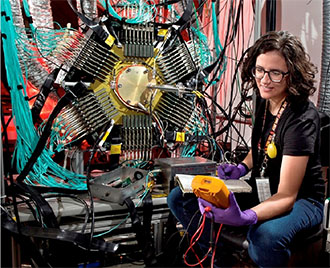
Photo. Verena Kleinrath with the fission Time Projection Chamber, which she uses to perform this work as part of her PhD thesis at Vienna University of Technology.
In the 1950s, Lab researchers first demonstrated that the strong anisotropies exhibited in neutron-induced fission depend on the incident neutron energy and fissioning nucleus. The results, generated by measuring the fragment anisotropy of several isotopes for incident neutron energies up to 10 MeV, had implications for cross-section measurements and understanding the underlying physics of the fission process. Now, a novel instrument at the Los Alamos Neutron Science Center (LANSCE) directly measures systemic fission effects that had previously been only calculated theoretically.
Neutron-induced fission cross sections are typically measured as ratios. Isotopes that exhibit different levels of anisotropy introduce a systematic error to the measurements. The angular distributions of the fragments are also closely related to the quantum mechanical state of the fissioning nucleus at the time of scission (where the nucleus breaks apart into two fragments). This phenomenon provides a rare window into the quantum world of the fissioning nucleus.
The Time Projection Chamber (TPC) project aims to measure the plutonium-239 fission cross section. The fission Time Projection Chamber (fissionTPC) tracks charged particles and their energy deposition. The unique instrument enables direct measurements of almost all of the systematic effects associated with the fission cross section, including the anisotropy. Previous cross section measurements typically corrected for anisotropy using theoretical calculations rather than obtaining it experimentally. The anisotropies of plutonium-239 and uranium-235 must be determined to achieve the goal of measuring the plutonium-239 fission cross section. Researchers have used the fissionTPC at LANSCE to make a significant advance toward measuring the plutonium-239 fission cross section to 1% accuracy.
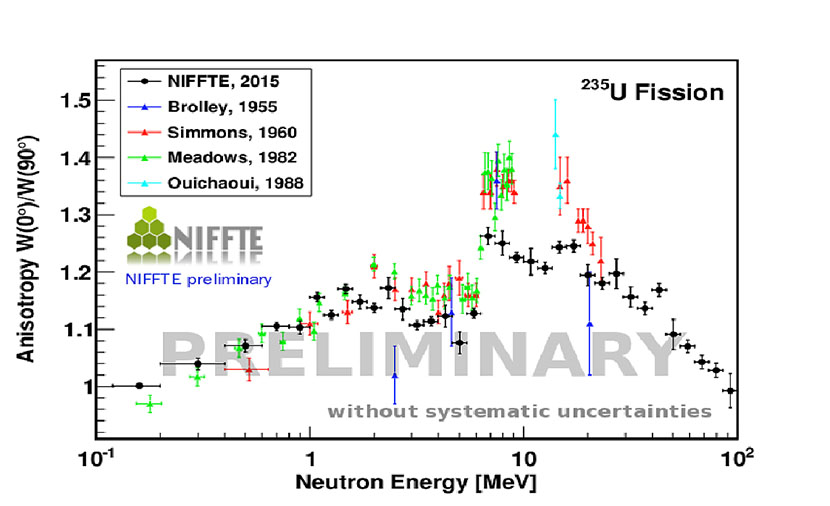
Figure 9. The preliminary results for the uranium-235 fission fragment anisotropy, defined as the emission probability of fission fragments in incident neutron beam direction over the probability of fragment emission perpendicular to the neutron beam. NIFFTE is Neutron Induced Fission Fragment Tracking Experiment.
Fredrik Tovesson, Brett Manning, Verena Kleinrath, and Dana Duke (LANSCE Weapons Physics, P-27) and collaborators measured the angular distribution of fission fragments from the neutron-induced fission of uranium-235. This achievement marks the first continuous measurement for incident neutron energies from 200 keV to several hundred MeVs. Preliminary results for uranium-235 fission emission anisotropy shown in Figure 9 generally agree with the energy dependence observed in previously measurements up to 10 MeV, although the absolute value is somewhat lower. Above 10 MeV the data are scarce, making meaningful comparisons difficult. After finalizing the results for uranium-235, the team plans to analyze plutonium-239 for which fewer data are available in the literature.
The fissionTPC measurements could also lead theorists to a better understanding of the fission process. The angular distributions provide insight into the transition states on top of the fission barriers as the compound nucleus deforms on its path to fission. More detailed information about those states would advance understanding of the fission process and allow scientists to more reliably calculate nuclear cross sections that cannot be measured directly.
Reference: “A Time Projection Chamber for High Accuracy and Precision Cross-section Measurements,” Nuclear Instruments and Methods in Physics Research A 759, 50 (2014); doi: 10.1016/j.nima.2014.05.057. Lawrence Livermore National Laboratory leads the TPC project. Authors’ institutions: Lawrence Livermore National Laboratory, Pacific Northwest National Laboratory, California Polytechnic State University, Idaho National Laboratory, Oregon State University, Ohio University, Colorado School of Mines, Idaho State University, Georgia Institute of Technology, Abilene Christian University, and Los Alamos National Laboratory (D. L. Duke, T. Hill, A. B. Laptev, R. Meharchand, L. Montoya, and F. Tovesson).
Science Campaign 1: Primary Assessment Technologies in the NNSA’s Science Campaign program funded this work as part of the fissionTPC project. The research supports the Laboratory’s Nuclear Deterrence mission area for stockpile stewardship and the Nuclear and Particle Futures science pillar. Technical contact: Fredrik Tovesson